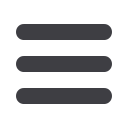
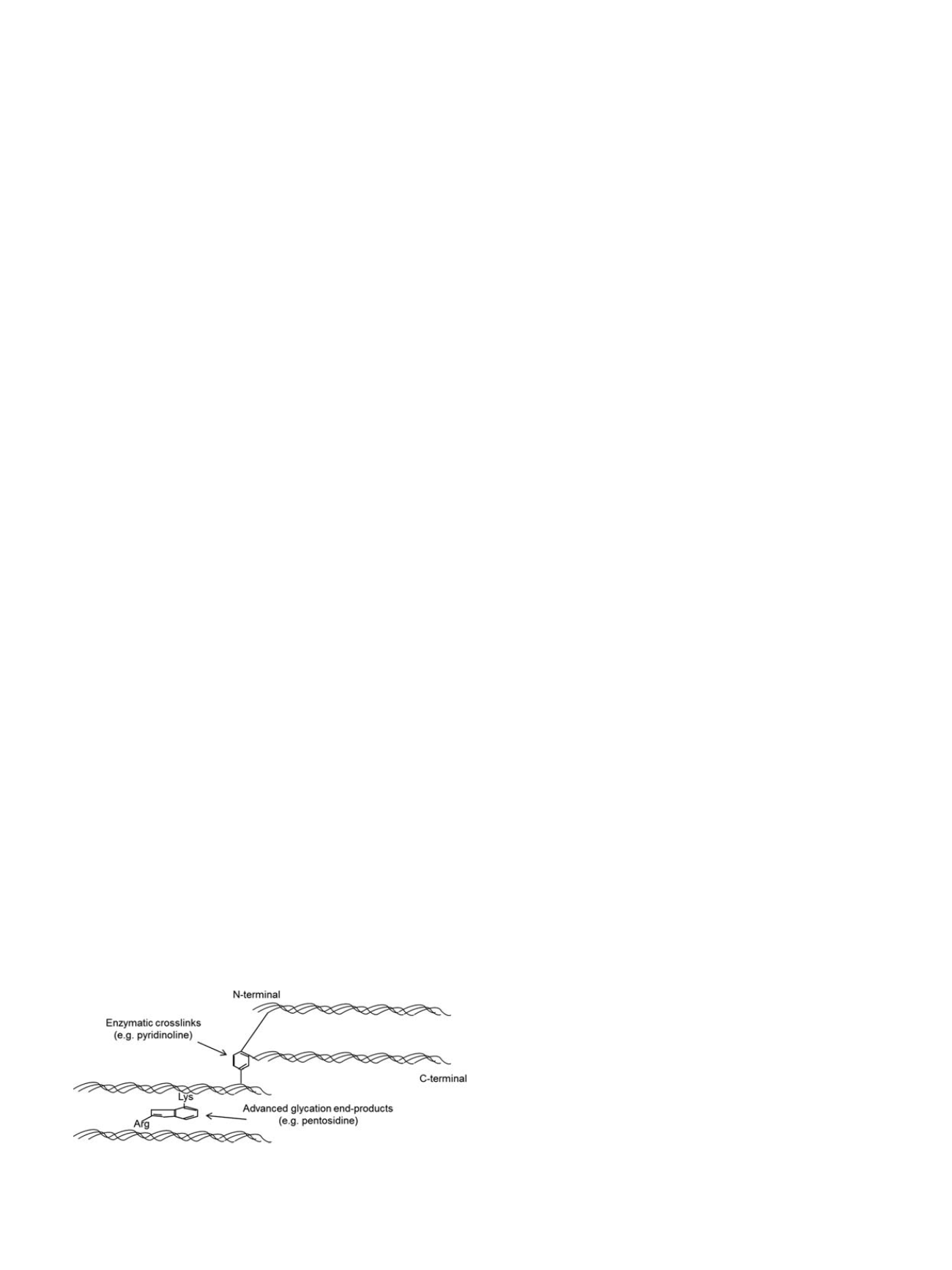
Although the crucial role of cortical bone for the mechanical
competence of bone and the risk of fracture has been recognized it has
not really been transferred to clinical practice for fracture risk
assessment or for monitoring of osteoporosis treatment. Future clinical
imaging techniques will have to consider measures cortical bone
geometrical features and also its local porosity.
The role of collagen
The matrix of bone is composed of both inorganic (i.e. mineral) and
organic (i.e. water, collagen, and non-collagenous proteins) compo-
nents. The role of mineral composition in skeletal fragility has been
studied in depth, and it is generally understood that in normal bone,
the mineral content provides strength and stiffness [81]. There is less
known about the effect of collagen and non-collagenous proteins, but
there is increasing evidence suggesting that changes in protein content
and structure play important roles in age- and disease-related changes
in bone. In particular, the organic matrix is considered to be
responsible for bone
’
s ductility and its ability to absorb energy prior
to fracturing [82].
Ninety percent of bone
’
s organic matrix is composed of type I
collagen, a structural protein comprised of three polypeptide chains
with a defined amino acid sequence, glycine-X-hydroxyproline or
glycine-proline-X (X is an amino acid such as lysine). This particular
sequence of amino acids allows the polypeptide chains to twist into a
triple helical structure with the small glycine in the middle, and amino
acids that remain exposed on the surface of the triple helix are involved
in the formation of collagen crosslinks [83]. Collagen undergoes
numerous post-translational modifications with aging and disease,
including both enzymatic and non-enzymatic crosslinking. In general,
enzymatic crosslinking is considered to be a normal process for healthy
collagen and has a beneficial effect on its mechanical properties, while
non-enzymatic crosslinking results in a brittle collagen network that
leads to deteriorated bone mechanical properties if its accumulation
exceeds normal repair [84].
Enzymatic crosslinking requires the enzyme lysyl oxidase to aid
the formation of intra- or inter-fibrillar crosslinks such as pyridinoline
and deoxypyridinoline [85]. The lysine-based crosslinks form in the
overlap regions of fibrils in a head-to-tail fashion (Figure 8) [86]. In
the maturation process, bivalent crosslinks slowly transform into a
more stable, trivalent, non-reducible conformation. Mature crosslinks
accumulate, inhibit collagen fibril remodeling, increase the stiffness of
the fibril, and provide increased strength to the tissue [86,87].
Pyridinoline and deoxypyridinoline serve as markers of bone resorp-
tion and are indicators of collagen maturity [88]. Enzymatic crosslinks
are most reliably quantified and characterized with mass spectrometry
[89] or HPLC (high performance liquid chromatography) [90], but some
studies indicate that FTIR (Fourier transform infrared) spectroscopy can
illustrate collagen crosslink characteristics [91]. Using these methods,
enzymatic crosslinks have been shown to be reduced in osteoporotic
patients with hip fractures compared to healthy controls [92,93].
The second pathway for collagen crosslinking does not involve any
enzymes, and is termed non-enzymatic glycation. Unlike the enzym-
atic crosslinks, which link the ends of the collagen molecules, non-
enzymatic crosslinks are found at any position along the collagen.
Non-enzymatic glycation involves a reaction between an aldehyde
group of a sugar (e.g. glucose) and the
ε
-amino group of hydroxylysine
or lysine. This reaction results in the formation of glucosyl-lysine,
which undergoes further reactions to form an Amadori product or
Schiff base adduct. Both of these intermediate products undergo
additional reactions to create crosslinks that form within and across
collagen fibers and are known as advanced glycation end-products
(AGEs) [86], which have been shown to accumulate in numerous
tissues including skin, cartilage, tendons, and bone [94]. AGEs
accumulate with age and disease [85]. Specifically, osteoporotic bone
has significantly more AGEs than normal healthy bone [92,93]. The
increased AGE levels can result in brittleness of tissues undergoing
non-enzymatic glycation [95].
There are two methods used for quantifying AGEs in bone, and
these techniques incorporate measurement of the autofluorescence
emitted by most AGEs. One technique quantifies pentosidine, a single
AGE crosslink and the only non-enzymatic crosslink that has been
successfully isolated and quantified in bone, using HPLC [96]. As
pentosidine composes less than 1% of total fluorescent AGEs in bone
and is weakly correlated to the amount of total fluorescent AGEs in
human bone [83,97], it is valuable to measure total fluorescent AGEs in
addition to pentosidine content. The second technique quantifies the
bulk fluorescence of AGEs from enzyme-digested or acid-hydrolyzed
bone samples relative to a quinine sulfate standard [98], and the
amount of fluorescence is normalized to collagen content.
Wavelengths used in this fluorometric assay capture the excitation
and emission wavelengths of several major AGE crosslinks including
pentosidine, carboxymethyllysine, vesperlysines, crossline, and car-
boxyethyllysine [83], and thus, the relative contributions of each of
these crosslinks to the total fluorescence cannot be determined from
this assay.
Increased non-enzymatic glycation has been shown to reduce
mechanical strength and/or toughness of bone [99,100]. Glycation
levels have also been shown to be greater in cadaver specimens from
hip fracture patients compared to controls, and the glycation content
was correlated with several biomechanical properties in cancellous
bone, but
not
in cortical bone [92,93]. Although it is generally
understood that AGEs accumulate in bone, stiffen the collagen
matrix, and in turn, deteriorate bone
’
s mechanical properties, the
contradictions in current literature arise for a number of reasons: (1)
few
in vitro
glycation studies have been conducted, and most
in vitro
studies have been primarily conducted in cancellous bone, (2) studies
conducted on
in vivo
glycation levels report pentosidine content only
while a few studies report total AGEs, making the studies difficult to
compare, (3) range of values for glycation levels reported vary greatly
depending on the bone, location, and age range of specimens used, and
(4) various mechanical testing techniques, animal models, or disease
states have been used in these studies. Thus, the exact contribution of
AGEs to age-related skeletal fragility remains undefined.
There is increasing evidence that AGEs directly affect cellular
function through the receptor for AGE (RAGE), a surface receptor on
many cell types [101]. RAGE activation is associatedwith inflammation,
cellular dysfunction, and localized tissue destruction. In bone,
activation of the RAGE receptor inhibits osteoblast proliferation and
differentiation [102], reduces matrix production [103], reduces bone
formation [104] and increases osteoblast apoptosis [105]. This
indicates that crosslinking properties of the matrix not only alter the
tissue properties, but directly control cellular function and may play
an important role in the decreased bone formation found in
osteoporosis [106].
In addition to enzymatic and non-enzymatic modifications of
collagen, non-collagenous proteins (e.g. osteopontin, osteocalcin),
Fig. 8. Collagen cross-links
A schematic illustration of enzymatic crosslinks (e.g. pyr-
idinoline [PYD], deoxypyridinoline [DPD]) and non-enzymatic crosslinks (e.g. pento-
sidine [PEN]) at the molecular level.
G. Osterhoff et al. / Injury, Int. J. Care Injured 47S2 (2016) S11
–
S20
S17