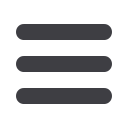
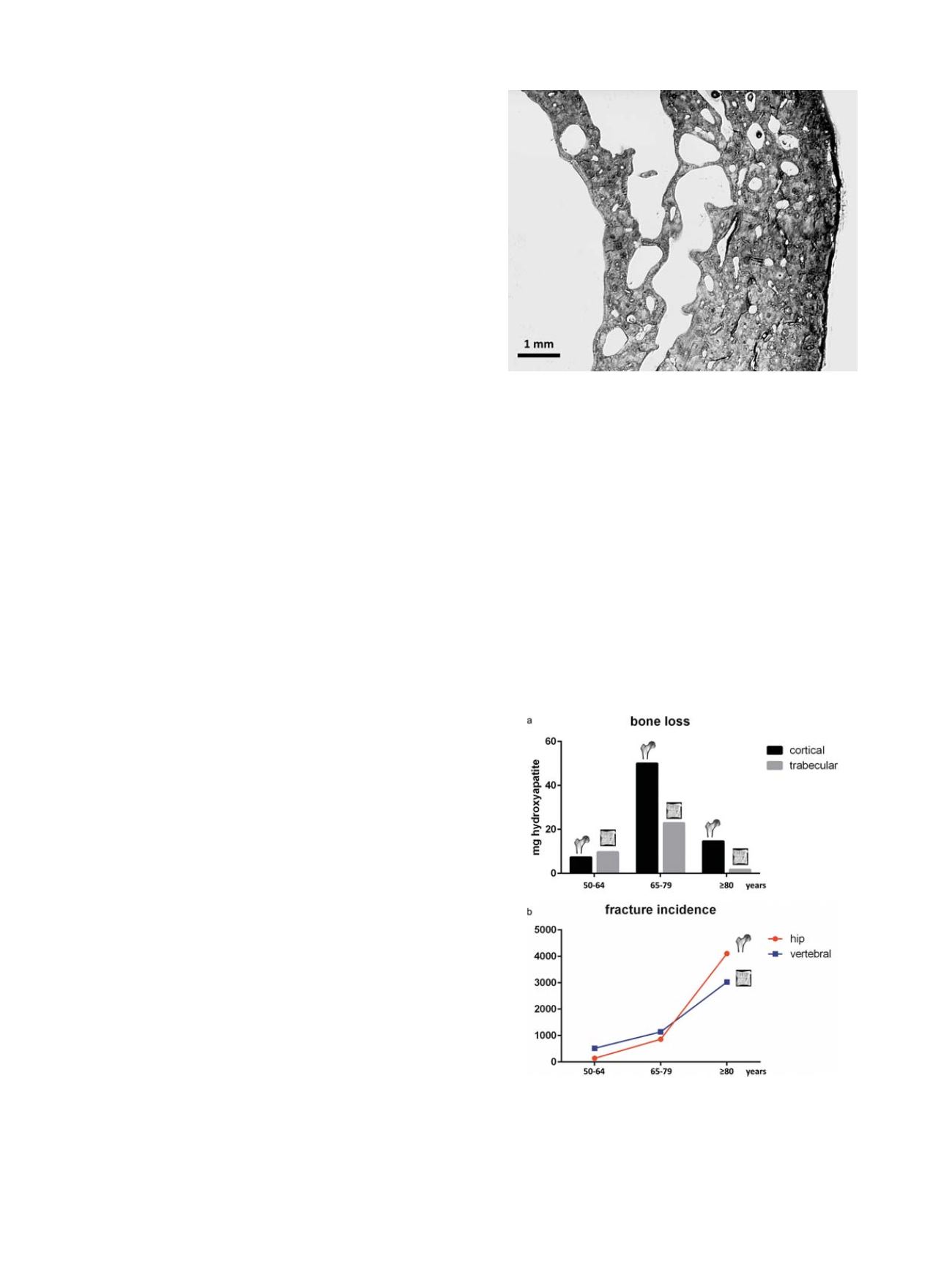
bone is supplied by diffusion from the surrounding bone marrow;
there are no vessels within trabeculae. Trabecular bone is always
surrounded by a cortical bone but the thickness and strength of the
cortical shell depends on location. Long bones, for example, show a
higher cortex-to-trabecular bone volume ratio than vertebrae and the
diaphyseal areas of long bones show a higher cortex-to-trabecular
bone ratio than the metaphyseal areas [10].
Cortical bone is stiffer and able to resist higher ultimate stresses
than trabecular bone, but it is also more brittle [10,13,14]. Trabecular
bone
in vitro
can withstand strains up to 30%, cortical bone fails with
strains of only 2%.While the biomechanical behaviourof cortical bone is
rather uniform, trabecular bone shows a wide variability in strength
and stiffness. This variability to the largest part depends on the
trabecular bone
’
s apparent density. Due to its heterogeneity, the
apparent density and thus the trabecular bone modulus can vary 100-
fold from one location to another within the same metaphysis [14].
Besides apparent density, stiffness and strength of cortical and
trabecular bone depend on the loading direction, indicating its
anisotropic microstructure [10,15,16]. In general, bone can resist
to higher compression loads than tension loads and to higher
tension loads than shear loads [15,16]. In line with this, the trabecular
connectivity inside a bone
–
as a measure of anisotropy
–
contributes
more to the bone
’
s biomechanical strength than the trabecular
thickness or the bone mineral density [17].
The mechanical response to loading, differs widely between
cortical and trabecular bone. Cortical bone, for instance, shows small
load carrying capacity when loaded beyond its range of elastic
deformation (post-yield) both with tensile and compression loads
[10,14]. In contrast, the load carrying capacity of trabecular bone is
insignificant after tensile fracture, but even larger than for cortical bone
after compressive fracture [14,18].
Each bone
’
s location in the body and the forces acting on it
determine its characteristic microstructure and composition. For
example, vertebral bodies must resist high and repetitive axial
compression loads but experience much less shear or tension loads.
If the trabecular bone is removed from a vertebral body, this leads to
increased cortical shell stresses and a disproportionate decrease in the
vertebral bone
’
s ability to withstand compression forces [19].
The femoral neck or the proximal humerus, on the other hand, is
mainly subjected to shear forces and bending moments, the latter of
which create a combination of compression, tension, and shear. Both
show a distinct cortical structure. There is only little change in the
biomechanical strength if the trabecular components are removed
from a proximal femur [20], but any reduction in cortical thickness or
change in cortical shape can increase the risk for sustaining a hip
fracture [21] or a proximal humerus fracture [22].
In vivo,
bone experiences different loads from different directions
and in different intensity and frequency over time. Bone has two main
structural responses to changing loading patterns: altering structural
density and increasing the degree of structural orientation along the
acting force vectors, i.e. anisotropy [10,14].
These adaptive responses would not be possible without the
existence of continuous bone remodelling. In bone remodelling,
bone tissue is removed by osteoclastic resorption and new bone is
formed by osteoblasts. In the early life span after skeletal maturity the
amounts of bone removed and replaced with each cycle of bone
remodelling are usually equal to each other, leaving the total volume of
bone unchanged. With ageing and in the setting of osteoporosis, the
balance of bone resorption and formation becomes negative. The bone
loss in aged and osteoporotic bone is a consequence of imbalanced and
excessive bone remodelling [11].
As bone remodelling occurs on osseous surfaces, osteoporotic bone
loss is a function of surface available for bone remodelling [23].
In individuals less than 65 years of age, the largest surface available
for bone remodelling is the trabecular bone. In this population,
trabecular bone
–
due to its lesser density when compared to cortical
bone
–
provides only about 20% of the skeletal bone mass but it is
responsible for most of the turnover [10,13]. Thus, the bone loss in early
osteoporosis is mainly a trabecular bone loss. With increasing age, the
cortical bone becomes more and more porous and, therefore, its
endocortical surface increases (Figure 1). As a consequence, the largest
loss of absolute bone mass due to osteoporosis occurs in corti-
cal bone by intracortical rather than endocortical or trabecular
remodelling [23].
The transition from early trabecular to later cortical bone loss is
consistent with the epidemiological data on osteoporotic fractures.
Vertebral compression fractures, being
“
trabecular fractures
”
, are more
common in individuals aged less than 65 years [24]. With increasing
cortical bone loss after the age of 65 years, hip fractures, being rather
“
cortical fractures
”
, become more frequent (Figure 2).
Fig. 1. Cortical bone trabecularization
Trabecularization of cortical bone at the
endocortical aspect of the cortex. Light microscopy of a quadrant of a female (age 91
years) femoral cortex at midshaft level.
Fig. 2. Association between bone loss and fracture incidence
(a) Cortical and tra-
becular bone loss in different age groups as shown by Zebaze et al. [20]. Early bone
loss occurs in the trabecular bone, but with increasing age the bone loss becomes
mainly cortical. (b) Incidence of osteoporotic hip and vertebral compression fractures
in different age groups in Switzerland as shown by Svedbom et al. [21]. Vertebral
compression fractures are more common in individuals aged less than 65 years. With
increasing cortical bone loss after the age of 65 years, hip fractures become the most
frequent entity.
G. Osterhoff et al. / Injury, Int. J. Care Injured 47S2 (2016) S11
–
S20
S12